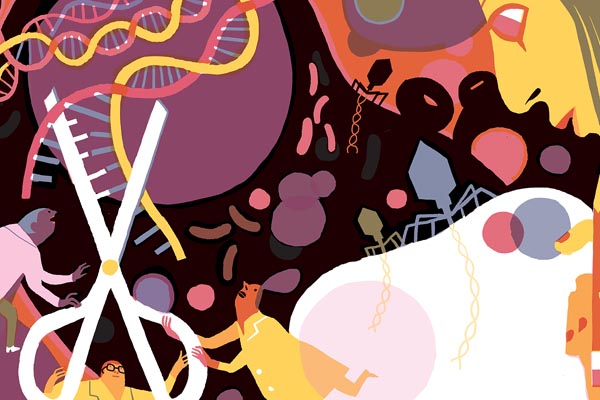
In a matter of weeks, Jeffrey Rathmell, PhD, and his research team can probe hundreds of genes and identify the ones that matter most in a particular disease model — and might be promising therapeutic targets.
Just a decade ago, such a strategy was simply not possible, said Rathmell, Cornelius Vanderbilt Professor of Immunobiology. But now there’s CRISPR (pronounced “crisper”), a genome editing technology that is simple and fast.
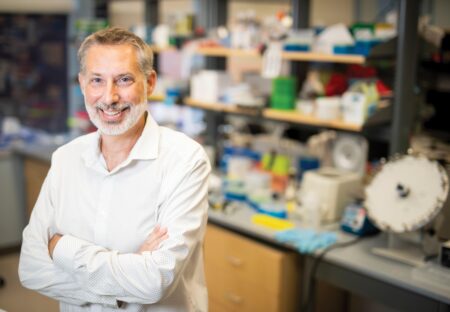
“What CRISPR allows us to do is change the genome with a great deal of efficiency and accuracy,” Rathmell said. “There were ways to make gene edits before, but they were challenging. It took a lot of effort to do one thing.
“In my view, CRISPR is in the top three advances in biology of the past hundred years. First, we understood what DNA was, then we sequenced the human genome, and now we can change it.”
CRISPR exploded onto the scene in 2012, with a publication in Science from the groups of Emmanuelle Charpentier, PhD, and Jennifer Doudna, PhD. Charpentier and Doudna shared the 2020 Nobel Prize in Chemistry for their genome editing discovery.
“It was just astounding to see how quickly it was possible to harness this as a tool for manipulating DNA in any cell type and whole organisms and by laboratories around the world,” said Doudna, winner of the 2020 Vanderbilt Prize in Biomedical Science, during a recent web-based Discovery Lecture for the Vanderbilt community. “It truly has been an enabling tool that has allowed scientists to do fundamental research and also to begin using it … in clinical medicine and agriculture and other areas of biology as well.”
Triggering repair processes
Charpentier and Doudna didn’t set out to develop a method for genome editing. They were in different parts of the world, pursuing their own interests in fundamental biological questions.
Charpentier and her team at the University of Vienna and then Umeå University in Sweden were studying how pathogenic bacteria cause disease and resist antibiotic therapy. Doudna and her group at the University of California, Berkeley were focused on the biological roles of RNA molecules.
Studies of how bacteria fight viral infections — using CRISPR — brought them together.
CRISPR is an acronym for “clustered regularly interspaced short palindromic repeats” — sequences of DNA found in many bacteria that are the hallmark of an adaptive immune system. The sequences mark a special spot in the genome where bacteria store pieces of DNA from infecting viruses. The CRISPR zone provides a template for the production of RNA molecules that combine with a DNA-cutting enzyme called Cas9 (CRISPR-associated protein 9) to form surveillance complexes that recognize and destroy matching viral DNA.
As they explored the molecular machinery and mechanism of the CRISPR-Cas9 system, the research teams discovered that it could be programmed to cut a specific DNA sequence. They knew that in plant and animal cells, a DNA cut would trigger repair processes that could introduce changes at the site of the cut.
A number of labs had recognized that introducing a double-strand DNA break would be a way to do genome editing, but the challenge had been “how do you cut the DNA at the place where you want the edit?” Doudna said.
CRISPR-Cas9 provided a solution: a programmable pair of molecular scissors.
“Nature figured out how to do gene editing, and we’ve now adopted nature’s approach,” said David Cortez, PhD, professor and chair of Biochemistry at Vanderbilt. “Our previous attempts were all engineering, and of course we’re not nearly as good as nature. Nature had millions of years to figure out how to do this; that’s why it works so well.”
Cortez, whose research focuses on DNA replication and repair, said it’s especially satisfying that studies in his field made an important contribution to harnessing the potential of CRISPR.
“If people hadn’t studied DNA repair, it wouldn’t have been possible to understand how CRISPR-Cas9 works and to use it for genome editing,” Cortez said. “The development of CRISPR really highlights the importance of curiosity-driven fundamental research.”
Game changer for research
CRISPR is now a common tool in research laboratories at Vanderbilt. The originally described CRISPR-Cas9 system usually inactivates genes because the repair machinery that stitches the DNA back together isn’t perfect and makes small deletions, Cortez said.
Investigators can delete a particular gene in cells or in mice and analyze what happens.
“This is really useful in the laboratory because you can inactivate genes and do loss of function analyses so easily,” Cortez said. “CRISPR is so much better than our previous methods of inactivating genes; it is much more targeted and efficient.”
Cortez and his team primarily use the strategy in cells to study the function of proteins involved in DNA replication and repair.
“CRISPR has been a complete game changer in terms of what we can do at the research level,” Rathmell said. He and his group inactivate batches of genes, dozens at a time, in pools of cells. They carefully control the conditions so that each cell has only one (or no) inactivated gene. Then they study the cells in culture or in animal models of disease and use sequencing to discover the genes that, when disrupted, give cells a fitness advantage or disadvantage.
“This CRISPR screening strategy lets us pick out genes that modify disease states and are potentially drug targets,” Rathmell said. “We’ve been able to generate a list of interesting targets very, very rapidly using CRISPR.”
The original CRISPR-Cas9 system comes from the bacterium Streptococcus pyogenes. But there isn’t just one enzyme anymore, said Mark Magnuson, MD, Louise B. McGavock Professor of Molecular Physiology and Biophysics.
“There are a lot of other prokaryotic microbes, and many have CRISPR-Cas systems that do different things,” said Magnuson, who directs the Vanderbilt Genome Editing Resource (VGER). “CRISPR is not just a tool chest with a hammer; there is now a whole set of different tools with unique molecular activities.”
VGER has assisted investigators in the generation of germline-altered mouse models since the early 1990s and has used CRISPR since 2013 to produce over 125 CRISPR-edited mice for more than 55 investigators.
Among the models are mice that have led to new insights about the genes that affect organ development and mice with glowing green epidermal growth factor receptors, the target of drugs used to treat colorectal, head and neck, and lung cancer.
VGER generally focuses on mouse models, but its associate director, Leesa Sampson, PhD, is happy to offer advice and help investigators design CRISPR strategies for cells and other model systems.
“The tool kit keeps changing and becoming better and better,” Sampson said. “We work with CRISPR all the time, and I still find out new things every day.”
Altering genes to treat disease
While CRISPR has transformed biomedical research, it is also making its way into the clinic, where it offers the prospect of cures for inherited diseases.
Two of the first genetic diseases to be treated with a CRISPR-based strategy are beta thalassemia and sickle cell disease. Both of these blood diseases are caused by mutations in the beta-globin gene, which codes for one of the subunits of hemoglobin. Patients with beta thalassemia do not make enough hemoglobin and have anemia. Patients with sickle cell disease have “sickle” shaped red blood cells that block blood vessels, causing severe pain and increasing the risk of organ damage and strokes.
A CRISPR strategy that has been tested in a small number of patients doesn’t attempt to fix the faulty gene. Instead, it increases production of fetal hemoglobin, which is normally turned off after birth. Blood-forming stem cells have been collected from patients, modified with the CRISPR treatment, and returned to patients.
In early results, the treated patients have near-normal hemoglobin levels that include 30 to 40% fetal hemoglobin. Bone marrow samples reveal cells with the expected genetic edit, and side effects have been limited to the bone marrow transplant chemotherapy regimen.
CRISPR treatments are also being tested for cancer (by altering tumor-killing T cells) and other diseases, and the tools are being used to diagnose COVID-19.
As potential genome editing treatments advance, investigators agree that there are concerns about off-target genome modification and unintended changes to the target site, along with issues related to the efficiency and delivery of the editing machinery.
With continuing improvements to existing and newly discovered CRISPR-Cas systems, worries about specificity will shrink, Rathmell said. “I think the concern about making unwanted changes that will lead to some other issue down the line is a very surmountable barrier.”
Efficiency and delivery challenges can be addressed by choosing the “right” diseases to treat with CRISPR, Rathmell added. Diseases that affect accessible cell populations like the blood are good choices, he said, as are organs like the lungs, where a potential treatment for cystic fibrosis might be introduced as an aerosol.
Heritable human genome editing
Beyond using CRISPR-based methods to correct disease-causing mutations in nonreproductive somatic cells, a large question looms. What about human genome editing that can be inherited — changes to human eggs, sperm or embryos?
“That’s not a future fantasy. It’s here,” Doudna said. “We’re not going to put that genie back in the bottle.”
Indeed, a Chinese scientist asserted in 2018 that he had used CRISPR to edit human embryos, to make them resistant to HIV, and that twin girls had been born. The stunning news of these “CRISPR babies” was roundly condemned, and the researcher was sentenced to three years in prison.
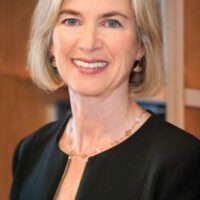
Scientists have convened international summits and issued reports and recommendations for heritable human genome editing. Doudna is on the organizing committee for the third international summit, which will be held in London next year.
“One of the keys to ensuring responsible use is having transparency around what’s happening, who’s doing what with the technology,” she said.
To that end, the World Health Organization has launched a Human Genome Editing Registry to collect information on clinical trials using human genome editing technologies. While the international scientific community agrees that it would currently be irresponsible to proceed with human germline genome editing, such technologies offer tantalizing opportunities to correct disease-causing mutations in a way that removes them from a family’s lineage.
“Might we one day conclude that that would be actually the best, the most ethical way to treat a disease? It’s possible,” Doudna said. “We have to just continue to evaluate the technology, but more importantly, to have transparent public discussion about it.”