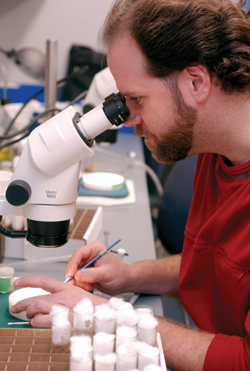
Kendal Broadie, Ph.D., evaluates mutant fruit flies under the microscope in his MRBIII laboratory. Dana Johnson
Brain’s plasticity modeled
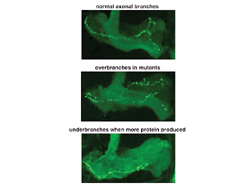
Nerve cell axonal projections from the brain of a normal fly (left), a fly with a mutant Fragile X protein (center), and a fly with overexpression of the Fragile X protein. Mutants show increased branching and excessive growth, whereas over-expression of the protein causes loss of branching and reduced growth. Courtesy Kendal Broadie, Ph.D.
Part 4 of 4
You may wonder why someone would study a fly to understand mental retardation, a decidedly human problem. But to Kendal Broadie, Ph.D., who studies the biology of the brain at its most basic level, it’s a uniquely powerful system.
“Drosophila melanogaster — the fruit fly — has a long history of being used as a genetic system to investigate biological questions,” said Broadie, professor of Biological Sciences. “You can define the area you’re interested in and just mutate the heck out of it until you find the genes that drive the process. Then you study them systematically until you’ve built up the picture. It’s like building a jigsaw puzzle.”
Broadie is deputy director of the Program in Developmental Neurobiology and Plasticity at the Vanderbilt Kennedy Center for Research on Human Development. The program is distinct from the other Kennedy Center research programs in its focus on the basic biology underlying developmental disorders of the brain.
The common thread connecting investigators within the program is an interest in understanding how brain circuitry develops and functions. Critical to that function is how malleable — or plastic — the brain is in adapting to change.
“The reason we can use animal models to study the basic mechanisms for how the brain works is that they are conserved across all species,” said Pat Levitt, Ph.D., professor of Pharmacology and director of the Vanderbilt Kennedy Center. “You can investigate how proteins interact and how that’s fundamental to synapses forming and stabilizing. Whether you do that in a fly, a mouse, or a monkey, you can get to these fundamental processes.”
Broadie’s research focuses on the synapse, the specialized junction between the brain’s cells that is the site of communication.
“By determining what cells partner to make a synapse and how strong that synapse is, you determine the pathways by which information flows in the brain,” he said. “That, in turn, determines everything else.”
Synaptic plasticity is believed to drive behavioral change, says Broadie.
“The idea is that activity initiates a change in synaptic communication, and that’s what we call learning,” he said. “If that change is hardwired, we call that memory.”
Using Drosophila, it’s possible to screen for genetic mutants defective in learning and memory, and then study their synaptic defects to infer the normal roles for the genes involved.
In his research, Broadie primarily works on three stages of the life cycle of the synapse: how it’s made, how it works, and how it changes throughout its life. An increasing interest in his laboratory is how synaptic function goes awry in neurological diseases, such as certain childhood-onset neurodegenerative diseases and Fragile X disease, the most common inherited form of mental retardation.
Broadie and his colleagues developed a Drosophila model to study how Fragile X defects occur. “We knew what the gene was and we had a good idea what it might be doing in a basic biological context,” he said. “With the model, we made what I think is a pretty significant understanding of the molecular basis of Fragile X disease that gives some hope of devising ways to treat it.” [See sidebar.]
In a similar way, Levitt uses the mouse model in his research to study how the brain is “wired up” and what the functional consequences are when things go wrong.
“The hope is that you can take that basic information and translate it into an intervention that gets things back on track,” he said.
One example is the work he does on interneurons, the cells in the brain’s cortex that put the brakes on activity.
“If you have too much activity, you have epilepsy and seizures,” said Levitt. “You need the inhibitory effect of the interneurons to prevent that.”
It turns out, Levitt says, that not only are seizures common in developmental disabilities — for example, about one third of children with autism have epilepsy — but so are anxiety and social disorders, which also seem to be associated with interneuron dysfunction.
“These kinds of neurons help regulate the way that the cerebral cortex integrates information — sort of putting the world together,” he said. “Autism is discussed sometimes as though the world is in its ultimate fragmented state.”
Levitt has been working with a mouse that has problems with the development of these interneurons.
“The mice develop epilepsy, they have anxiety disorders, and it looks like they might have social interaction disorder,” he said. “We’ve identified the neurons that get disrupted, and we think that the cells may not move properly from their place of origin to their final location during development — they get sort of stalled out.”
If that translates to humans, Levitt says, it might be possible to figure out how to get the neurons “on the right track” to their final resting position. Or, to find something that would raise inhibitory activity in the absence of those neurons.
“This is an example of basic developmental neurobiology,” said Levitt. “How do these cells get to their right location, how do they integrate and make a circuit, and what are the molecules involved? Knowing that gives us a way of testing different strategies — using a drug or stem cells or cell transplants, for instance — to make up for the fact that a specific population of cells is missing.”
Levitt and Broadie have plans to recruit new researchers to join them and others at Vanderbilt working in the area of neurocircuitry and synaptic biology, such as Randy Blakely, Ph.D., and Elaine Sanders-Bush, Ph.D., in Pharmacology, Danny Winder, Ph.D., and Roger Colbran, Ph.D., in Molecular Physiology and Biophysics, and Ford Ebner, Ph.D. in Psychology and Cell and Developmental Biology.
Other areas of investigation targeted for expansion are human neurogenetics — studying the genetic basis of disorders of brain development that lead to mental retardation or to neuropsychiatric disorders — and auditory system development and plasticity.
Vanderbilt is very strong in the sensory sciences, says Levitt, mostly vision, thanks to people like Jeff Schall, Ph.D. in Psychology, and Jon Kaas, Ph.D. in Psychology and Cell & Developmental Biology, who also studies somatosensation (touch).
Levitt would like to recruit other researchers to join Troy Hackett, Ph.D., a basic neuroscientist with appointments in the departments of Psychology and Hearing and Speech Sciences who studies the auditory system.
“If you think about it, the one sensory science that would link beautifully to our strengths in clinical research — for example, we have very strong language and communication and reading people — would be the auditory system,” he said.
“We’re building a community of people,” said Broadie. “It doesn’t matter what department you’re in, what matters is that there is a community of people who work closely together on related issues that all support the mission of the Kennedy Center. If we hire the right people, this place could really become a center for this kind of research.”
Fly guides researcher to fruition
by Mary Beth Gardiner
Using the fruit fly to study the underlying cause of Fragile X syndrome, the most common inherited form of mental retardation in humans, may appear surprising at first glance.
But the short reproductive cycle and easily mutated genome of the tiny fly makes it a powerful tool for Kendal Broadie, Ph.D., professor of Biological Sciences, to use in exploring the neurobiology of the disease.
When he first set his mind to the task, Broadie, who is also deputy director of the Program in Developmental Neurobiology and Plasticity at the Vanderbilt Kennedy Center for Research on Human Development, already knew what gene was disrupted in Fragile X syndrome. He even had some idea of the role of the protein encoded by the gene — evidence suggests it binds to messenger RNA inside the cell and regulates whether or not other proteins are expressed, or translated.
“The complexity is that the protein apparently binds to hundreds of different messenger RNAs,” said Broadie. “So in the absence of the protein — as occurs in Fragile X syndrome — you potentially mistranslate hundreds of different proteins. How do you even begin to think of a way around that?”
In tackling the genetics of the problem in the fruit fly, Broadie discovered that the RNA message of a particular protein — called microtubule associated protein 1b, or MAP1b — is normally bound by the Fragile X protein. But in the absence of the Fragile X protein, MAP1b is over-expressed and the cell’s microtubules, which are responsible for transportation of various molecules into and out of the cell, malfunction.
“What’s so amazing is that if you correct that single problem, all the defects seen in the mutant flies go away,” said Broadie.
It’s too early to conclude that the same effect might occur in humans. However, the finding is exciting, Broadie says, because it links Fragile X to inappropriate stabilization of the microtubule skeleton in nerve cells. And drugs that modulate microtubule stability are already used in humans to treat a number of problems, including cancer.
Broadie is also pursuing this work in a mouse model of Fragile X, but he says the experiments won’t be easy. “The defects in mice are pretty subtle,” he said. “If you were another mouse, you’d say, ‘That guy’s got a problem.’ But if you’re a human looking at that mouse, you might think, well it eats, it runs — it does what a mouse does.”
Still, Broadie is hopeful that his work in flies will lead to new directions in the mouse model. “A lot of what I did has already been replicated in the mouse,” he said.
“Showing that MAP1b is normally down-regulated by the Fragile X protein has been replicated, and so has showing that levels of MAP1b go up in the absence of Fragile X protein. But the experiment where I corrected the defects in the flies — we don’t have the technology to do that in the mouse. Not yet, anyway.”